As more natural landscapes are becoming urbanized in South Carolina, proper stormwater conveyance is needed to protect natural water resources. Urbanization is linked with increased surface water pollution and erosion. At the same time, there is an increasing demand for clean water as the population grows. Planners and municipal managers are encouraged to install low impact development (LID) strategies to meet these needs. Managers, planners, and residents will benefit from understanding the associated concerns of urbanization and two of the most basic LID strategies: bioretention cells and stormwater wetlands. While bioretention cells promote water infiltration, stormwater wetlands provide water retention.
Introduction
Impervious areas, including asphalt, concrete, buildings, and even highly compacted soil surfaces, cover natural landscapes where water cannot infiltrate.1,2,3 With an increase in urbanization in the United States and worldwide, impervious surfaces are increasing and are expected to continue increasing.3,4,5 Impervious surfaces directly affect the hydrology of an environment. For example, as the number of impervious surfaces (addition of roads, parking lots, and buildings) increases, infiltration is reduced, increasing surface runoff.3 Other issues associated with increased impervious areas include reduced groundwater recharge, decreased water quality, and degraded environments.3 Stream ecosystems begin to show evidence of degradation when impervious areas make up 10% of a watershed.3 When impervious coverage reaches 30% or more, the watershed is considered severely degraded and, in most cases, beyond the scope of practical restoration efforts.6,7 For example, with the conversion of natural lands for the development of the Seattle-Tacoma International Airport in the state of Washington, the 14 km2 watershed could no longer effectively detain the stormwater runoff.8 As a result, increased erosion, increased flooding frequency, degradation of fish habitat, and declined water quality were documented.8
Impervious surfaces cause a two-fold issue for the environment. First, most water deposited by rain on an impervious surface does not reach the natural soil surface.3 The water is instead collected as stormwater by a drainage system and channeled to either a wastewater treatment facility or into a convenient body of water.9,10 Where the stormwater ends up depends upon the region and the stormwater systems implemented. In South Carolina, any stormwater runoff that enters the drainage system will enter surrounding bodies of water untreated.11 Additionally, since impervious surfaces limit the volume of water infiltrating the natural soil, groundwater recharge is reduced.12,13 Further, a study in the Piedmont and Blue Ridge region of Georgia compared the streamflow characteristics of highly urbanized watersheds to those of less urbanized and non-urbanized watersheds and documented a notable decline in water levels in urban wells compared to non-urban wells.14 This supports the conclusion that impervious surfaces decrease groundwater recharge.14
The second issue with impervious surfaces is the increased surface runoff velocity and volume15, 16, leading to increased erosion.17 The increased velocities of surface runoff are generally not dangerous to people but may be detrimental to the environment. A sheet of water flowing off an impervious area (e.g., a parking lot) may erode topsoil from the receiving natural area. The soil and chemicals associated with soil (e.g., fertilizers, pesticides) are carried with the stormwater directly into local streams and rivers.3 The soil particles can stay suspended in the water column, creating a turbid (murky, opaque) environment.18 The suspended solids can change a waterbody’s physical, chemical, and biological properties, affecting aquatic life.19 For example, suspended solids can decrease the amount of light that penetrates the water, which is known to reduce the growth rate of primary producers such as algae, thereby reducing food sources for primary consumers.19 Suspended solids can also clog and damage fish gills.20 Along with soil particles, any chemical present on the impervious surface (e.g., oil, heavy metals, industrial products) may also enter receiving bodies of water.12,21 For example, lead, copper, cadmium, and zinc are common metals found in stormwater runoff emanating from building sidings and roofs.21 The heavy metals found in runoff from impervious surfaces can contaminate the water and become toxic to the ecosystem’s organisms if high enough concentrations are present.
The high velocities associated with stormwater runoff can impact the physical characteristics (e.g., water movement, water distribution, flow rate) of a water channel and the surrounding landscapes.15 Furthermore, higher volumes and velocities accelerate erosion, resulting in flow patterns and channel stability changes.8 Streams may widen or become deeper to accommodate the increased volume and velocity of the water.22 Eroded material from the streambank may also deposit and accumulate downstream, changing the morphology of the stream.23
Low Impact Development Water Management Strategies
In the past, conventional practices such as conveyance piping systems (figure 1a) were commonly used for stormwater management.24 Conventional practices collect water runoff through drains (figures 1a, 1b) and route the stormwater via corrugated metal pipe or steel-reinforced polyethylene pipe (figures 1c, 1d, 1e). The water can be routed directly off-site to a municipal plant or receiving waterways and indirectly through dry and wet stormwater detention basins (figures 2a, 2b, 2c, 2d).25 Conventional practices do not utilize soft engineering or engineering that mimics natural processes (e.g., infiltration, evaporation).
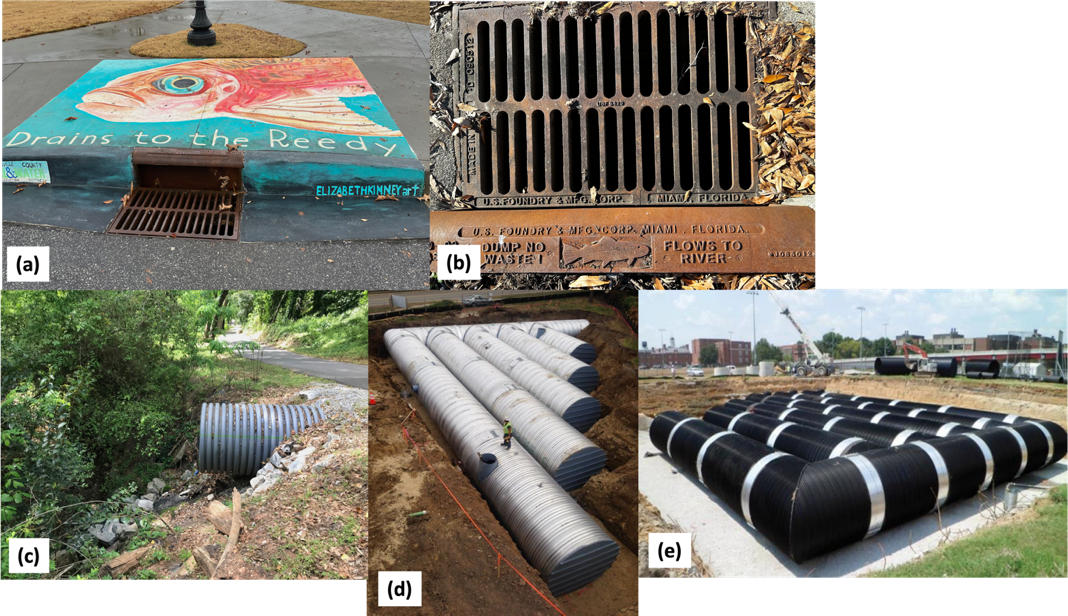
Figure 1. Conventional stormwater management includes utilizing drains that go directly to a waterway (a, b). Typically, drains are labeled. Large conveyance pipes move stormwater to its next destination (c, d, e). Image credit: Heather Nix, Clemson Cooperative Extension (a, e); James Lenhart, Stormwater Northwest LLC (b, d); Dara Park, Clemson Cooperative Extension (c).
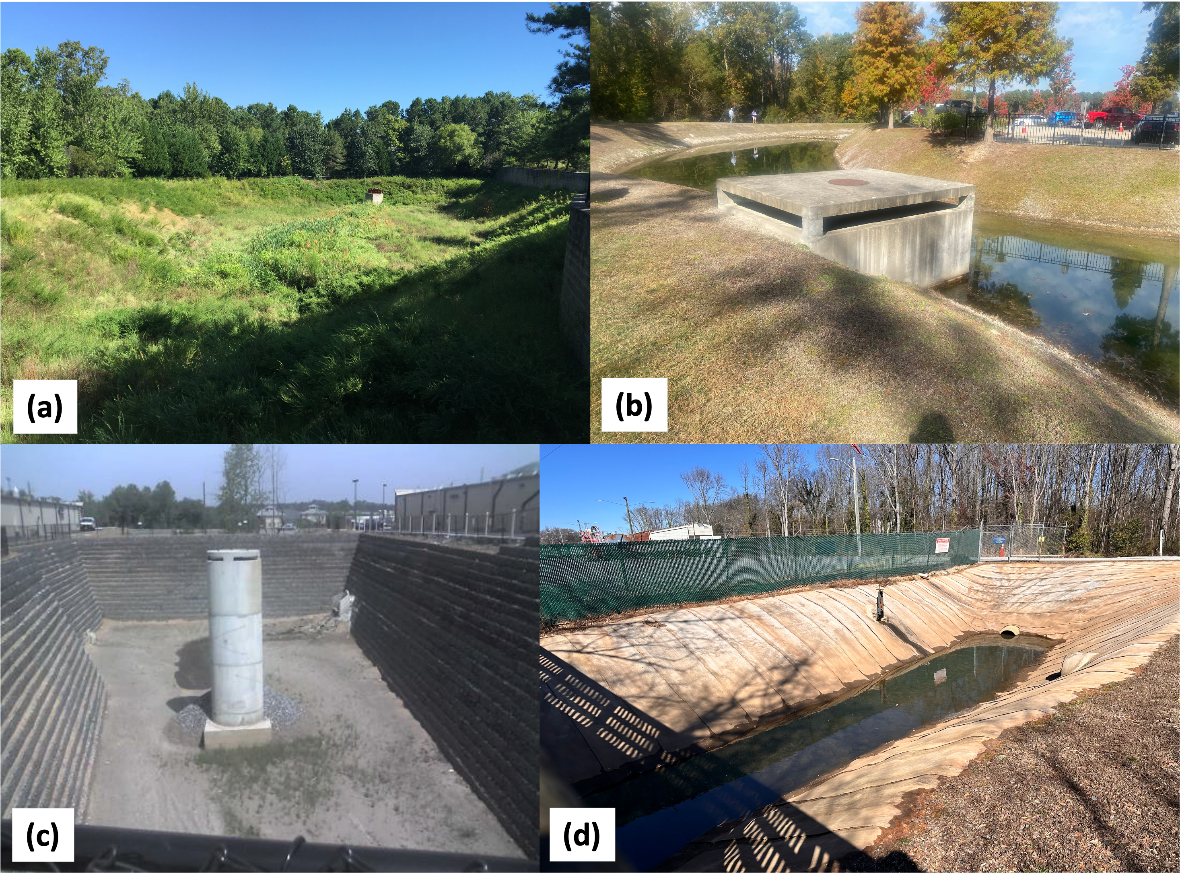
Figure 2. Conventional stormwater management systems utilized dry attenuation cells (a) or wet attenuation cells (b) before being discharged to the treatment plant or waterway. Conventional stormwater detention basins are built with impervious surfaces such as cement (c, d). Image credit: Sarah Waickowski, North Carolina State University (a, b); James Lenhart, Stormwater Northwest LLC (c); Dara Park, Clemson Cooperative Extension (d).
Soft engineering integrates natural ecological principles and processes with existing infrastructure to help manage stormwater and conserve environmental services.26,27 Examples of soft engineering include using buffer strips and natural or constructed wetlands.28 Buffer strips use vegetation to help decrease erosion and protect surface water bodies from diffuse pollution.28 The incorporation of soft engineering into urban development is called low impact development (LID).29 LID strategies improve and sustain natural resources (e.g., water, energy, air). Stormwater LID strategies aim to restore stream functions and ecosystem integrity in and surrounding urbanized environments to pre-development hydrologic conditions.29, 30
Currently, swales are the most common type of stormwater conveyance integrated into built environments.31 While there are different types of swales, including infiltration swales, bioswales, wet swales, and grass swales (figures 3a, 3b, 3c), grass swales are the most common.32 Swales are soft engineering as they make use of vegetation, such as grasses, to enhance natural hydrological components of infiltration and evapotranspiration.33
Two other water management LID practices that implement soft engineering are bioretention cells and constructed wetlands. Bioretention cells and constructed wetlands take advantage of natural water treatment processes and are designed to reduce peak runoff rates following storm events and improve water quality.34 To demonstrate this, a study in North Carolina documented how similar flow rates of undeveloped watersheds are to the outflows of a bioretention cell.35 Stormwater wetlands and bioretention cells may look similar, but their engineering and function differ.

Figure 3. Examples of swales include grass swales seen in the foreground of (a) and bare swales seen in the background of (a), recently mowed swale (b), and infiltration swale (c). Image credits: Heather Nix, Clemson Cooperative Extension (a, c) and Sarah Waickowski, North Carolina State University (b).
Bioretention Cells
Bioretention cells collect stormwater runoff and allow water to infiltrate into and drain through the soil (figure 4).34 The design aims to reduce runoff volumes into natural stream systems and assists with water percolating into surrounding soils and recharging groundwater.34 The design of a bioretention cell includes highly porous engineered soil media, vegetation, and organic materials such as mulch (figure 5).36 Typically, the vegetation selected for bioretention cells is resilient to environmental stress, such as widely varying soil moisture conditions, temporary submergence and drier periods.34 Along with promoting a more natural system, vegetation helps to trap sediments and reduces runoff velocity.37 Infiltrated water can also exit the cell if an underdrain is present.36 Underdrains are perforated pipes installed beneath the soil media, and a layer of gravel is typically included where the underlying soil has low permeability.38
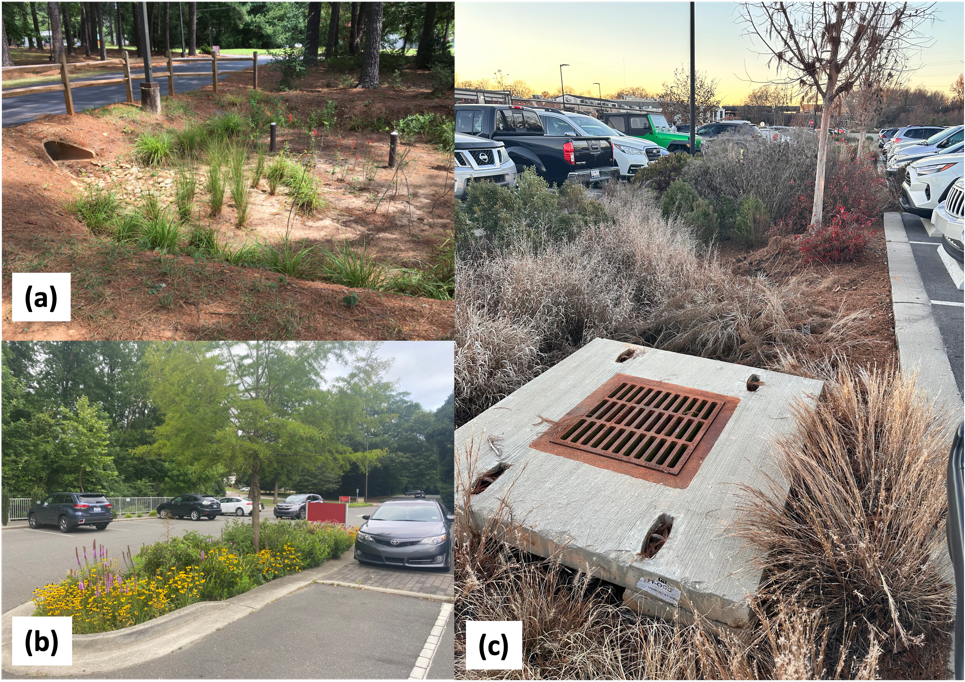
Figure 4. Various bioretention cells: newly planted with inlet on left (a) and smaller bioretention cells integrated into parking lots (b, c). Image credits: Sarah Waickowski, North Carolina State University (a, b) and Dara Park, Clemson Cooperative Extension (c).

Figure 5. Bioretention cell schematic. Image credit: North Carolina Department of Environmental Quality Stormwater Design Manual C-2.39
There is no single way to design a bioretention cell, as different environments and climates pose different challenges.36 For example, regions with extreme winters must account for snow melt, frozen soil, and freeze-thaw cycles.36 Specifically, the design of bioretention cells may differ in the soil mediums used.38 The soil mediums used in developing a bioretention cell are critical to the functioning and efficiency of the system. Soil media physical properties influence the water movement, storage, and filtration capacity of a bioretention cell.38 The clay contents of the soil mediums are typically low (below 8%) to encourage infiltration.38
While bioretention cells can be low-maintenance stormwater solutions, sedimentation can reduce infiltration over time. Bioretention cells often require periodic cleaning to drain and perform optimally.38 Removal of sediment from the stormwater before the stormwater enters the bioretention cell is required to reduce cleanout frequency.38 It is also recommended to periodically add organic material, such as compost materials, to support plant growth.40 Monitoring the inflow and outflow of bioretention cells will help identify how the system’s performance is sustaining over time.41
Due to the limited number of studies investigating the function of bioretention cells over extended periods of time, the longevity of bioretention cells is not well established.42, 43 However, current guidelines suggest that the lifespan of a bioretention cell that receives maintenance is between twenty and twenty-five years before soil media needs to be replaced and/or rehabilitation is needed.40 Paus et al. (2014) explored the functioning of bioretention cells from 2006 to 2010. The results of this study suggest that bioretention cells can provide long-term (> six years) effective infiltration.44 Additionally, Johnson and Hunt (2019) monitored the water quality of a bioretention cell in North Carolina sixteen years after the initial analysis.45 Johnson and Hunt found that mass load reductions of total nitrogen and total phosphorous both increased after seventeen years, indicating that bioretention cells can continue to be efficient at removing nutrients from the stormwater runoff is the bioretention cell is maintained.45
Constructed Stormwater Wetlands
Stormwater wetlands, also called constructed wetlands, are typically shallow, vegetative areas that store runoff water and release the water.34 Constructed stormwater wetlands are engineered to help protect from floods, improve water quality, and promote diversity.46 Constructed stormwater wetlands are developed with two important parts: a forebay and a macrophyte zone (planted or vegetated zone) (figure 6). These zones include shallow water (0 ft to 1.5 ft deep) and deep water (1.5 ft to 6 ft deep). When stormwater reaches the wetland, it should first enter a forebay, where most sediment will settle out before reaching the macrophyte zone. When the water flows into the macrophyte zone, the remaining sediment will be trapped and settled near the wetland plants.47 The macrophyte zone is composed of various plants, which aid in absorbing nutrients and toxic substances.48 To see a detailed schematic and learn more about stormwater wetland design, refer to chapter C-4 of the “North Carolina Department of Environmental Quality Stormwater Design Manual” (https://bit.ly/3vEv3Kj).49 Compared to bioretention cells, which are designed to infiltrate water into the soil and recharge groundwater, the purpose of a constructed wetland is to retain water.
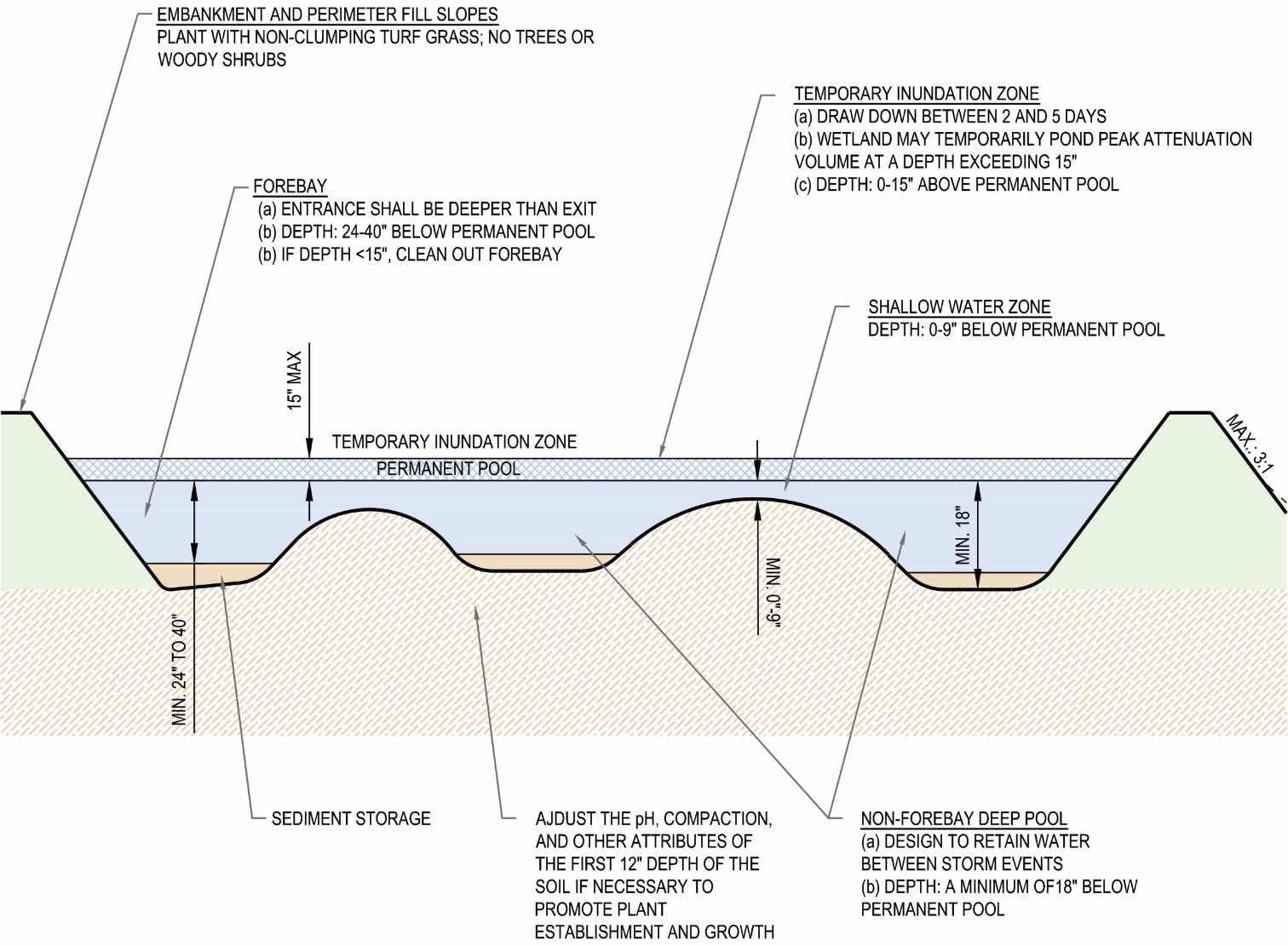
Figure 6. Stormwater wetland schematic. Image credit: North Carolina Department of Environmental Quality Stormwater Design Manual C-4.48
There are three basic types of constructed wetlands (figures 7a, 7b, 7c, 7d, 7e): free water surface (surface flow) wetlands, subsurface flow wetlands, and hybrid wetlands.50 Free water surface wetlands resemble shallow marshes with water standing on the surface. In contrast, a subsurface flow wetland resembles a horizontal bioretention cell where the objective is to filter water by moving it through a constructed media rather than vegetation, as in a free water wetland. Hybrid wetlands have characteristics of both free water and subsurface water wetlands and are commonly used for wastewater that is difficult to remediate.50 For more information on the types of constructed wetlands, please refer to the “Handbook of Constructed Wetlands” (https://bit.ly/47E9V3Y).51 Additionally, the South Carolina Department of Health and Environmental Control (SCDHEC) defines five different wetland applications: (1) stormwater wetland, (2) shallow wetland, (3) extended detention shallow wetland, (4) pond/wetland system, and (5) pocket wetland. Each wetland application is different in design and purpose.52 For more information, refer to the Stormwater Wetlands chapter (https://bit.ly/4aKLZhp) in SCDHEC’s “Storm Water Management BMP Handbook”.52
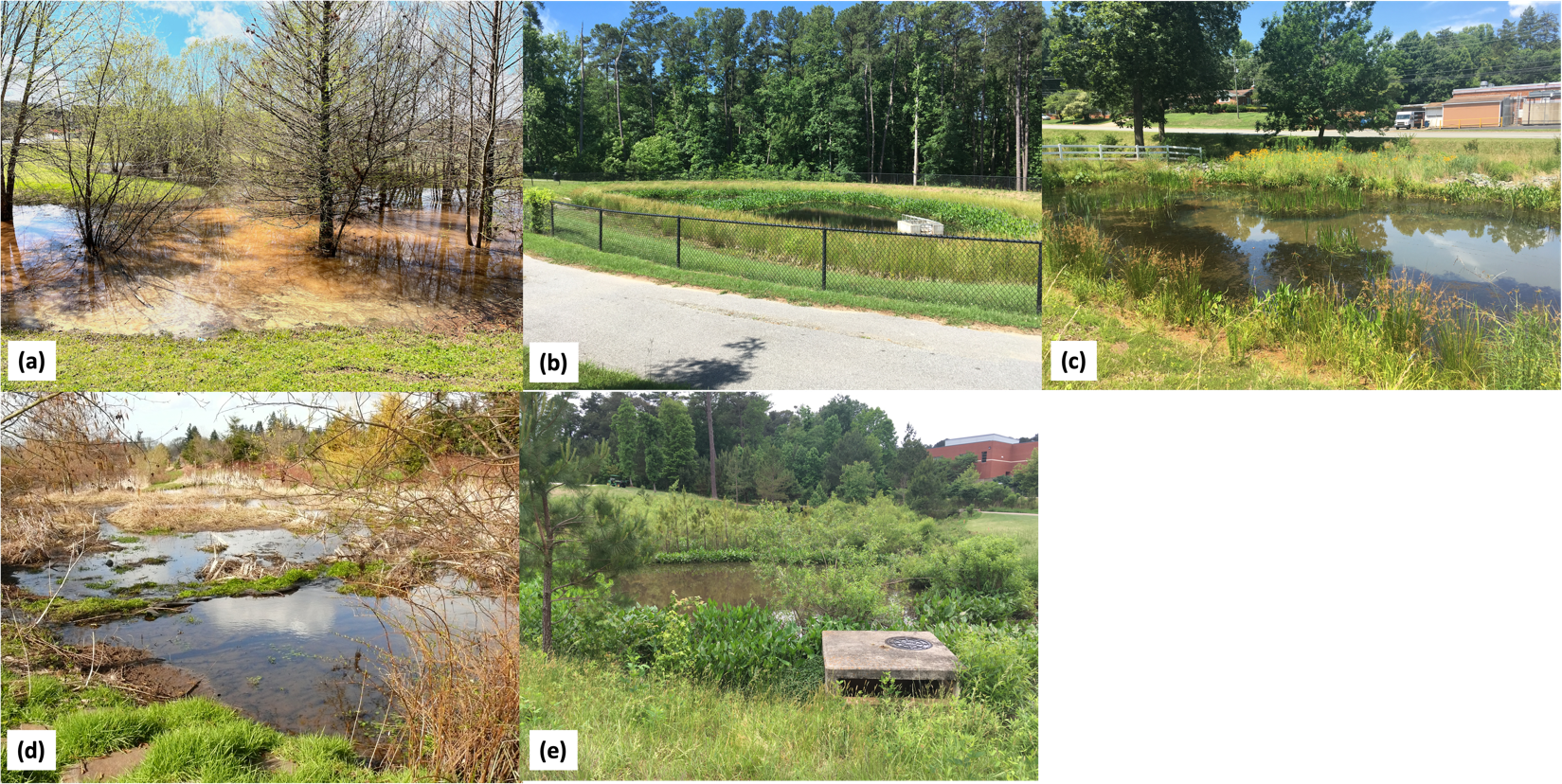
Figure 7. Constructed free water surface stormwater wetlands can have trees (a), only herbaceous vegetation (b and c), or vegetation with different statures (d, e). Image credits: Heather Nix, Clemson Cooperative Extension (a); Sarah Waickowski, North Carolina State University (b, c, e); and Sarah White, Clemson Cooperative Extension (d).
The construction and establishment of stormwater wetlands can take two years or more; this longer duration can be viewed as a negative undertaking.47 However, constructed wetlands have a relatively low, long-term cost compared to conventional treatment systems, which can be seen as positive.48 Constructed wetlands systems require large surface areas to attain desired treatment efficacy, typically between 1% and 2% of the watershed area.53 Depending on the watershed size, several acres of land may need to be acquired, contoured, and extensively planted. A significant investment (time and money) in initial plant establishment in the wetlands is required to establish healthy stands of wetland vegetation. Natural plant colonization and succession may take years. Maintenance of a constructed wetland includes cleaning out retained sediments caught in the sediment forebay when sediment levels reach 500 mm below the normal water level.47 Constructed wetlands are known to function efficiently long-term.54
Plant Selection
Plant selection is essential when developing LID strategies such as bioretention cells and constructed wetlands.55 Prioritizing plant diversity in some LIDs, such as bioretention cells, has been recommended to increase performance,56 including removal of specific contaminants.55 Regardless of the type of LID strategy implemented, plant selections must suit the expected soil hydrology.55 For constructed wetlands, native plants typically found in wetlands should be selected as these plants are adapted to the wetting and drying cycles of a wetland.57 For bioretention cells, select plants with a deep, extensive root structure.58 It is also recommended to include plants with dense linear foliage and a spreading growth pattern in bioretention cells, as these plant characteristics aid in preventing soil erosion.58 Navigate to North Carolina Department of Environmental Quality chapters on bioretention cells and stormwater wetlands (https://www.deq.nc.gov/about/divisions/energy-mineral-and-land-resources/stormwater/stormwater-program/stormwater-design-manual) ) for plant suggestions and other resources on how to find appropriate native plants.39, 49
Site Considerations for Stormwater Bioretention Cells and Constructed Wetlands
LID strategies are commonly used with other stormwater management practices, including green urban technologies such as green roofs, permeable pavements, vegetated medians, and greenways (figure 8).59 By incorporating multiple strategies, LID aids in various management functions and follows a systematic approach. For example, green roofs, bioretention cells, curb-less roads with swales, and permeable pavements can all be used in an urban environment for stormwater controls.29 If properly designed, an urban area using LID principles can minimize contaminant transport and soil erosion while increasing the neighborhood’s aesthetic appeal.29
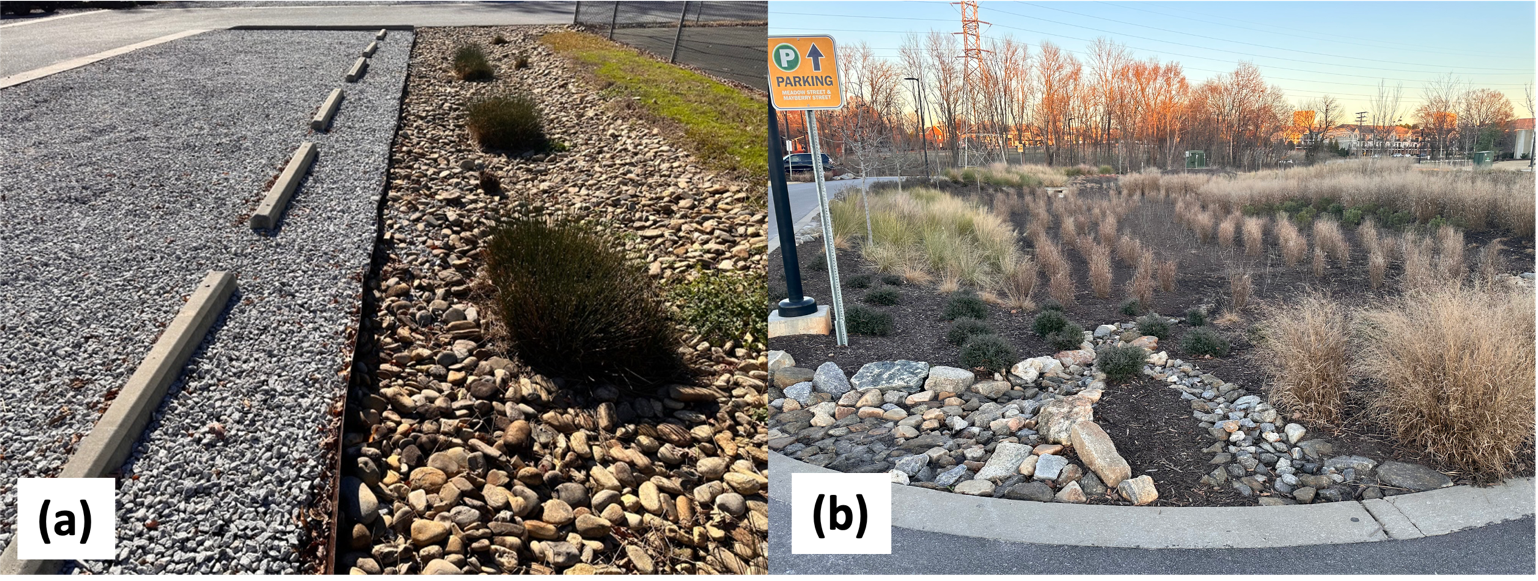
Figure 8. Examples of incorporating green technology with LID strategies to enhance stormwater management: pervious gravel parking lot and vegetative infiltration swale (a) and curb-less road leading into rock inlet into large bioretention cell as part of a park landscape (b). Image credit: Dara Park, Clemson Cooperative Extension.
New developments should be designed incorporating LID strategies and soft engineering. Determining which potential LID strategy is appropriate is based on different characteristics of the proposed site:
- Location: Where the LID practice will be installed is one of the most important variables influencing the system’s effectiveness.60 The area where the LID strategy is to be installed must be suitable. The suitability of an area is based on land use, spatial scale, and position in the stream network.61 Depending on municipal regulations, the area needed for stormwater management will be determined by the area of impervious surfaces. Regulations will specify if and how the stormwater management areas can be divided. Generally, bioretention cells require less land area than stormwater wetlands.38 The US guidelines recommend the catchment area of a bioretention cell to be under two acres (0.8 ha) as increased erosion and discharge are associated with larger catchment areas.62
- Landscape topography: The landscape’s topography will affect the layout and shape of LID installations.62,63 For example, if the topography is steep, bioretention cells may be built on terraces to limit the potential of erosion.59 The slope of the land near the LID infrastructure (such as bioretention cells) should be at most 5%, as steep slopes promote higher water velocities, increasing erosion.62
- Soil: Soil will determine how the stormwater moves over the land surface and how water infiltrates through the soil. A soil’s hydrologic soil group describes the permeability and runoff potential of the soil.64 This term is defined by the Natural Resource Conservation Service (NRCS) based on rainfall, runoff, and infiltration data. The lower the infiltration rate is, the higher the runoff potential. The USDA’s online Web Soil Survey Tool (https://bit.ly/4bb6tBd) can be used to understand the soil’s hydrologic group.
- Proximity of water resources: Depending upon the topography and soil hydrologic group, the depth to the seasonal high water table may need to be determined.65 For example, when implementing a bioretention cell, at least two feet of separation is needed between the bottom of the cell and the seasonal high water table.65 Additionally, if groundwater contamination is a concern, an impervious layer should be incorporated into the bottom of the cell.65 Adding an impervious layer to the bioretention cell will help to prevent the stormwater from leaching into the groundwater. Taking these measures into account helps to decrease the potential for groundwater contamination.65 This information can also be found by utilizing the Web Soil Survey Tool (https://bit.ly/4bb6tBd).
- Identifying anticipated contaminants: The possible and anticipated contaminants within stormwater should be identified before implementing a LID strategy.62 Knowing the anticipated contaminants will help identify what plants to incorporate, as some plants are better adapted to remove specific contaminants (e.g., nutrients and metals) than others.62
In general, water-focused LID strategies are implemented with the goal of reducing peak stormwater discharge rates and volumes to natural surface water bodies. The complexity of land uses and site characteristics within a watershed result in multiple potential types and locations for applying LID strategies.66 Decision support tools like the one developed by Liu et al. (2016) may be used to select, identify, and place LID practices within an environment to optimize environmental benefits while minimizing costs.66 In addition, bringing in a professional engineer or landscape architect is essential to make sure the best management decisions are made for the environment in the most economical means.
Are Bioretention Cells and Stormwater Wetlands Effective at Managing Stormwater Runoff?
Actual performance values of bioretention cells and stormwater wetlands vary depending on the type of evaluation performed (e.g., flow rate, contaminant removal), but research documents that bioretention cells and stormwater wetlands can be highly effective if implemented correctly. A report commissioned by the Minnesota Department of Transportation reports a median phosphorous removal rate of 42% for stormwater wetlands and 72% for bio-retention cells.67 The median suspended sediment removal rate was 68% for stormwater wetlands and 90% for bioretention cells.67 The North Carolina Department of Environmental and Natural Resources states that bioretention cells may remove an average of 60% of total nitrogen depending on filter media, and stormwater wetlands are credited with eliminating an average of 40% of total nitrogen.68 A publication by the USEPA estimates that bioretention cells may remove up to 98% of metals (Cu, Zn, Pb) and 90% of bacteria.69 The USEPA documents an average removal rate of 50% for the same metals and a 77% removal rate for bacteria from stormwater wetlands.70 Generally speaking, bioretention cells effectively remediate contaminants such as total suspended solids, heavy metals, nitrogen, phosphorus, E. coli, and fecal coliform in stormwater.71
A recent study by Dutta et al. (2021) documented that bioretention cells and vegetative swales improved water quality by removing total suspended solids, total nitrogen and total phosphorus and effectively reducing peak flow.72 In addition, the study concluded that using bioretention cells, vegetative swales, and porous pavements in combination with each other was more efficient than using a single practice.72
Performance measures, including water runoff volume and velocity reduction, depend highly on the LID design and construction and the maintenance program. Still, these studies illustrate that LID strategies can remove contaminants effectively.
Conclusion
Stormwater management is an essential concern with the continual increase of impervious surfaces and urbanization throughout South Carolina. The effects of erosion and pollutants found in stormwater directly impact the quality of water and water supplies people rely on, as well as the surrounding environments and ecosystems. Public demand must increase for the use of LID-based water management strategies within developments to protect and improve water quality. Whether as a homeowner choosing to channel runoff from home roofs to a 100 sf bioretention cell or as a civil engineer designing a multi-acre wetland to receive runoff from a commercial park. The use of LID practices is an essential part of effectively managing stormwater and protecting its quality.
References Cited
- Arnold Jr C, Gibbons C. Impervious surface coverage the emergence of a key environmental indicator. Journal of the American Planning Association. 1996 Jun [accessed 2023 Dec 22];62(2):243–258. doi:10.1080/01944369608975688.
- Lu D, Weng Q. Use of impervious surface in urban land-use classification. Remote Sensing of Environment. 2006, May [accessed 2023 Dec 22];102(1-2):146–160. doi:10.1016/j.rse.2006.02.010.
- Chithra S V, Nair M H, Amarnath A, Anjana N S. (2015). Impacts of impervious surfaces on the environment. International Journal of Engineering Science Invention. 2015 May [accessed 2023 Dec 22];4(5):27–31. https://www.academia.edu/download/37942361/E045027031.pdf
- Elvidge C, Tuttle B, Sutton P, Baugh K, Howard A, Milesi C, Bhaduri B, Nemani R. Global distribution and density of constructed impervious surfaces. Sensors. 2007 Sep [accessed 2023 Dec 22];7(9):1962–1979. doi:10.3390/s7091962.
- Theobald D, Goetz S, Norman J, Jantz P. Watersheds at risk to increased impervious surface cover in the conterminous United States. Journal of Hydrologic Engineering. 2009 Apr [accessed 2023 Dec 22];14(4):362–368. doi:10.1061/(ASCE)1084-0699(2009)14:4(362).
- Kloster T, Leybold T, Wilson C. Green streets: innovative solutions for stormwater and stream crossings. Global Solutions for Urban Drainage. 2002 [accessed 2023 Dec 22]:1–18. doi:10.1061/40644(2002)1.1.
- Rames L. Watershed impairment in Buncombe County, North Carolina: impervious groundcover relations to stream degradation. 2016 NCUR. 2016 Jul [accessed 2023 Dec 22]. https://libjournals.unca.edu/ncur/wp-content/uploads/2021/06/1689-Rames-Samantha-FINAL.pdf.
- Booth D, Hartley D, Jackson R. Forest cover, impervious‐surface area, and the mitigation of stormwater impacts. JAWRA Journal of the American Water Resources Association. 2002 Jun [accessed 2023 Dec 22];38(3):835–845. doi:10.1111/j.1752-1688.2002.tb01000.x.
- Barbosa A, Fernandes J, David L. Key issues for sustainable urban stormwater management. Water Research. 2012 Dec [accessed 2023 Dec 22];46(20):6787–6798. doi:10.1016/j.watres.2012.05.029.
- Bonneau J, Fletcher T, Costelloe J, Poelsma P, James R, Burns M. Where does infiltrated stormwater go? Interactions with vegetation and subsurface anthropogenic features. Journal of Hydrology. 2018 Dec [accessed 2023 Dec 22];567:121–132. doi:10.1016/j.jhydrol.2018.10.006.
- What is Stormwater? Columbia (SC): South Carolina Department of Health and Environmental Control; 2019 [accessed 2023 Dec 22]. https://scdhec.gov/what-stormwater.
- Klein R. Urbanization and stream quality impairment. JAWRA Journal of the American Water Resources Association. 1979 Aug [accessed 2023 Dec 22];15(4):948–963. doi:10.1111/j.1752-1688.1979.tb01074.x.
- Pasquier U, Vahmani P, Jones A. Quantifying the city-scale impacts of impervious surfaces on groundwater recharge potential: an urban application of WRF–Hydro. Water. 2022 Oct [accessed 2023 Dec 22];14(19):3143. doi:10.3390/w14193143.
- Rose S, Peters N. Effects of urbanization on streamflow in the Atlanta area (Georgia, USA): a comparative hydrological approach. Hydrological Processes. 2001 Jun [accessed 2023 Dec 22];15(8):1441–1457. doi:10.1002/hyp.218.
- Jacobson C. Identification and quantification of the hydrological impacts of imperviousness in urban catchments: A review. Journal of Environmental Management. 2011 Jun [accessed 2023 Dec 22];92(6):1438–1448. doi:10.1016/j.jenvman.2011.01.018.
- Bera D, Kumar P, Siddiqui A, Majumdar A. Assessing impact of urbanisation on surface runoff using vegetation-impervious surface-soil (VIS) fraction and NRCS curve number (CN) model. Modeling Earth Systems and Environment. 2021 Jan [accessed 2023 Dec 22]:1–14. doi:10.1007/s40808-020-01079-z.
- Doyle M, Harbor J, Rich C, Spacie A. Examining the effects of urbanization on streams using indicators of geomorphic stability. Physical Geography. 2000 Mar [accessed 2023 Dec 22];21(2):155–181. doi:10.1080/02723646.2000.10642704.
- Mandal K. Assessment of wastewater temperature and its relationship with turbidity. Recent Research in Science and Technology. 2014 Oct [accessed 2023 Dec 22];6(1):258–262. https://core.ac.uk/download/pdf/236011105.pdf.
- Bilotta G, Brazier R. Understanding the influence of suspended solids on water quality and aquatic biota. Water research. 2008 Jun [accessed 2023 Dec 22];42(12):2849–2861. doi:10.1016/j.watres.2008.03.018.
- Wong C, Pak I, Jiang Liu X. Gill damage to juvenile orange‐spotted grouper Epinephelus coioides (Hamilton, 1822) following exposure to suspended sediments. Aquaculture Research. 2013 Oct [accessed 2023 Dec 22];44(11):1685–1695. doi:10.1111/j.1365-2109.2012.03173.x.
- Davis A, Shokouhian M, Ni S. Loading estimates of lead, copper, cadmium, and zinc in urban runoff from specific sources. Chemosphere. 2001 Aug [accessed 2023 Dec 22];44(5):997–1009. doi:10.1016/S0045-6535(00)00561-0.
- Bledsoe B, Watson C. Effects of urbanization on channel instability. Journal of the American Water Resources Association. 2001 Apr [accessed 2023 Dec 22];37(2):255–270. doi:10.1111/j.1752-1688.2001.tb00966.x.
- Tuttle R, Wenberg R. Streambank and shoreline protection. In: Engineering field handbook. Washington (DC). U.S. Dept. of Agriculture, Natural Resources Conservation Service; 1996. ENG- National Engineering Handbook (NEH), Part 650, Engineering Field Handbook (EFH).
- Ahiablame L, Engel B, Chaubey I. Effectiveness of low impact development practices: literature review and suggestions for future research. Water Air & Soil Pollution. 2012 Sep [accessed 2023 Dec 22];223:4253–4273. doi:10.1007/s11270-012-1189-2.
- McCarthy J. New Hampshire stormwater manual: volume 1 stormwater and antidegradation. Merrimack (NH): Comprehensive Environmental Inc; 2008 Dec. WD-08-20A. Funded in part through a Clean Water Act Section 319 Nonpoint Source Program grant and a Clean Water Act Section 104(b)(3) grant from the U.S. Environmental Protection Agency.
- Shannon K. Eco-engineering for water: From soft to hard and back. Resilience in Ecology and Urban Design: Linking Theory and Practice for Sustainable Cities. 2012 Dec [accessed 2023 Dec 22];3:163–182. doi:10.1007/978-94-007-5341-9_8.
- Hewett C, Wilkinson M, Jonczyk J, Quinn P. Catchment systems engineering: a holistic approach to catchment management. Wiley Interdisciplinary Reviews: Water. 2020 May [accessed 2023 Dec 22];7(3):e1417. doi:10.1002/wat2.1417.
- Fogg P, King J, Shepherd M, Clemence B. A review of ‘soft engineering’ techniques for on-farm bioremediation of diffuse and point sources of pollution. London (GB): Department for Environment, Food and Rural Affairs (DEFRA) Science Division; 2005.
- Eckart K, McPhee Z, Bolisetti T. Performance and implementation of low impact development–a review. Science of the Total Environment. 2017 Dec [accessed 2023 Dec 22];607:413–432. doi:10.1016/j.scitotenv.2017.06.254.
- Islam A, Hassini S, El-Dakhakhni W. A systematic bibliometric review of optimization and resilience within low impact development stormwater management practices. Journal of Hydrology. 2021 Aug [accessed 2023 Dec 22];599:126457. doi:10.1016/j.jhydrol.2021.126457.
- Burian S, Edwards F. Historical perspectives of urban drainage. Global Solutions for Urban Drainage. 2002 [accessed 2023 Dec 22]:1–16. doi:10.1061/40644(2002)284.
- Currier B, Taylor M. California Department of Transportation BMP retrofit pilot program. Building Partnerships. 2004 [accessed 2023 Dec 22]:1–10. doi:10.1061/40517(2000)80.
- Ekka S, Hunt B. Swale terminology for urban stormwater treatment. Urban waterways. Raleigh (NC). NC State University Cooperative Extension. 2020 Feb [accessed 2023 Dec 22]. AG-588-26. https://content.ces.ncsu.edu/swale-terminology-for-urban-stormwater-treatment#:~text=Grass%20swales%20are%20historically%20the,(Currier%20and%20Taylor%202004).
- Roy-Poirier A, Champagne P, Filion Y. Review of bioretention system research and design: past, present, and future. Journal of Environmental Engineering. 2010 Sep [accessed 2023 Dec 22];136(9):878–889. doi:10.1061/(ASCE)EE.1943-7870.0000227.
- DeBusk K, Hunt W. Bioretention outflow: does it mimic rural water interflow? World Environmental and Water Resources Congress 2011: Bearing Knowledge for Sustainability 2011. 2011 [accessed 2023 Dec 22]:375–386. doi:10.1061/41173(414)41.
- Khan U, Valeo C, Chu A, He J. A data driven approach to bioretention cell performance: prediction and design. Water. 2013 Jan [accessed 2023 Dec 22];5(1):13–28. doi:10.3390/w5010013.
- DeBusk K, Wynn T. Stormwater bioretention for runoff quality and quantity mitigation. Journal of Environmental Engineering. 2011 Nov [accessed 2023 Dec 22];137(9):800–808. doi:10.1061/(ASCE)EE.1943-7870.0000388.
- Liu J, Sample D, Bell C, Guan Y. Review and research needs of bioretention used for the treatment of urban stormwater. Water. 2014 Apr [accessed 2023 Dec 22];6(4):1069–1099. doi:10.3390/w6041069.
- North Carolina Department of Environmental Quality. C-2. Bioretention cell. In: NCDEQ stormwater design manual. Raleigh (NC): North Carolina Environmental Quality; 2018 [revised 2018 Jan 19; accessed 2024 Feb 22].
- Spraakman S, Van Seters T, Drake J, Passeport E. How has it changed? A comparative field evaluation of bioretention infiltration and treatment performance post-construction and at maturity. Ecological Engineering. 2020 Dec [accessed 2023 Dec 22];158:106036. doi:10.1016/j.ecoleng.2020.106036.
- Cording A, Hurley S, Whitney D. Monitoring methods and designs for evaluating bioretention performance. Journal of Environmental Engineering. 2017 Dec [accessed 2023 Dec 22];143(12):05017006. doi:10.1061/(ASCE)EE.1943-7870.0001276.
- Wang M, Zhang D, Wang Z, Zhou S, Tan S. Long-term performance of bioretention systems in storm runoff management under climate change and life-cycle condition. Sustainable Cities and Society. 2021 Feb [accessed 2023 Dec 22];65:102598. doi:10.1016/j.scs.2020.102598.
- Vijayaraghavan K, Biswal B, Adam M, Soh S, Tsen-Tieng D, Davis A, Chew S, Tan P, Babovic V, Balasubramanian R. Bioretention systems for stormwater management: Recent advances and future prospects. Journal of Environmental Management. 2021 Aug [accessed 2023 Dec 22];292:112766. doi:10.1016/j.jenvman.2021.112766.
- Paus K, Morgan J, Gulliver J, Leiknes T, Hozalski R. Assessment of the hydraulic and toxic metal removal capacities of bioretention cells after 2 to 8 years of service. Water, Air, & Soil Pollution. 2014 Jan [accessed 2023 Dec 22];225:1–12. doi:10.1007/s11270-013-1803-y.
- Johnson JP, Hunt WF. A retrospective comparison of water quality treatment in a bioretention cell 16 years following initial analysis. Sustainability. 2019 Apr [accessed 2024 Feb 14];11(7);1945. doi:10.3390/su11071945.
- Dumax N, Rozan A. Valuation of the environmental benefits induced by a constructed wetland. Wetlands Ecology and Management. 2021 Dec [accessed 2023 Dec 22];29(6):809–822. doi:10.1007/s11273-021-09811-x.
- Gato-Trinidad S, Carroll J, Aladin E, Gilbert T. Understanding the role of constructed wetlands in stormwater management. In: Ferreira TF, Shi H, editors. Flood risk in a climate change context – exploring current and emerging drivers. London (GB): IntechOpen; 2022 Mar [accessed 2023 Dec 22]. doi:10.5772/intechopen.102912.
- Gopal B. Natural and constructed wetlands for wastewater treatment: potentials and problems. Water Science and Technology. 1999 Jan [accessed 2023 Dec 22];40(3):27–35. doi:10.1016/S0273-1223(99)00468-0.
- North Carolina Department of Environmental Quality. C-4. Stormwater Wetland. Raleigh (NC): North Carolina Environmental Quality; 2020 [revised 2020 Nov 20; accessed 2023 Dec 20]. https://www.deq.nc.gov/energy-mineral-and-land-resources/stormwater/bmp-manual/c-4-stormwater-wetland-11-20-2020/download
- Austin G, Yu K. Constructed wetlands and sustainable development. 1st ed. London (GB): Taylor & Francis Group; 2016. doi:10.4324/9781315694221.
- U.S. Environmental Protection Agency. A handbook of constructed wetlands. A guide to creating wetlands for agricultural wastewater, domestic wastewater, coal mine drainage, stormwater in the Mid-Atlantic Region. Volume 1 Washington (DC): U.S. Environmental Protection Agency; 2015.
- South Carolina DHEC. BPM Field Manual. Columbia (SC): South Carolina Department of Health and Environmental Control, Stormwater Management; 2005.
- Council M. Minnesota urban small sites BMP Manual: stormwater best management practices for cold climates. St Paul (MN): Metropolitan Council, Barr Engineering Company; 2001.
- Parde D, Patwa A, Shukla A, Vijay R, Killedar D, Kumar R. A review of constructed wetland on type, treatment and technology of wastewater. Environmental Technology Innovation. 2021 Feb [accessed 2023 Dec 22];21:101261. doi:10.1016/j.eti.2020.101261.
- Vogel J, Moore T, Coffman R, Rodie S, Hutchinson S, McDonough K, McLemore A, McMaine J. Critical review of technical questions facing low impact development and green infrastructure: a perspective from the Great Plains. Water Environment Research. 2015 Sep [accessed 2023 Dec 22];87(9), 849–862. doi:10.2175/106143015X14362865226392.
- Yuan J, Dunnett N, Stovin V. The influence of vegetation on rain garden hydrological performance. Urban Water Journal. 2017 Nov [accessed 2023 Dec 22];14(10):1083–1089. doi:10.1080/1573062X.2017.1363251.
- Wong T, Breen P, Somes N, Lloyd S. Managing urban stormwater using constructed wetlands. Clayton, Victoria (AU): Monash University, Cooperative Research Centre for Catchment Hydrology, Centre Office: Department of Civil Engineering; 1999. Industry Report, Report 98/7. 2nd ed.
- Hunt W, Lord B, Loh B, Sia A. Plant selection for bioretention systems and stormwater treatment practices. 1st ed. London (UK): Springer Nature, Springer Singapore; 2014. doi:10.1007/978-981-287-245-6.
- Duan H, Li F, Yan H. Multi-objective optimal design of detention tanks in the urban stormwater drainage system: LID implementation and analysis. Water Resources Management. 2016 Jul [accessed 2023 Dec 22];30:4635–4648. doi:10.1007/s11269-016-1444-1.
- Passeport E, Vidon P, Forshay K, Harris L, Kaushal S, Kellogg D, Lazar J, Mayer P, Stander E. Ecological engineering practices for the reduction of excess nitrogen in human-influenced landscapes: a guide for watershed managers. Environmental Management. 2013 Feb [accessed 2023 Dec 22];51:392–413. doi:10.1007/s00267-012-9970-y.
- Martin-Mikle C, de Beurs K, Julian J, Mayer P. Identifying priority sites for low impact development (LID) in a mixed-use watershed. Landscape and Urban Planning. 2015 Aug [accessed 2023 Dec 22];140:29–41. doi:10.1016/j.landurbplan.2015.04.002.
- Paus K, BrasKerud B. Suggestions for designing and constructing bioretention cells for a Nordic climate. Journal of Water Management and Research. 2014 [accessed 2023 Dec 22];3:139–150. https://www.researchgate.net/profile/Bent-Braskerud/publication/287121896_The_hydrological_performance_of_bioretention_cells_in_regions_with_cold_climates_Seasonal_variation_and_implications_for_design/links/5ced0df3458515026a614969/The-hydrological-performance-of-bioretention-cells-in-regions-with-cold-climates-Seasonal-variation-and-implications-for-design.pdf.
- Persson J, Somes N, Wong T. Hydraulics efficiency of constructed wetlands and ponds. Water Science and Technology. 1999 Jan [accessed 2023 Dec 22];40(3):291–300. doi:10.1016/S0273-1223(99)00448-5.
- NRCS. Part 630 hydrology. In: National engineering handbook. Washington (DC): United States Department of Agriculture, Natural Resource Conservation Service; 2001. 210–630–H.
- Clar M, Barfield BJ, O’Connor T. Stormwater best management practices design guide volume 1 – general considerations. Washington (DC): U.S. Environmental Protection Agency; 2004. EPA/600/R-04/121. https://cfpub.epa.gov/si/si_public_record_report.cfm?Lab=NRMRL&dirEntryId=99739.
- Liu Y, Cibin R, Bralts V, Chaubey I, Bowling L, Engel B. Optimal selection and placement of BMPs and LID practices with a rainfall-runoff model. Environmental Modelling & Software. 2016 Jun [accessed 2023 Dec 22];80:281–296. doi:10.1016/j.envsoft.2016.03.005.
- Weiss P, Gulliver J, Erickson A. The cost and effectiveness of stormwater management practices. In: Thurston HW, editor. Economic incentives for stormwater control. Boca Raton (FL): CRC Press, Minnesota Department of Transportation, Research Services Section; 2005 Jun 1. doi:10.1201/b11071.
- NC Department of Environmental Resources. Stormwater wetlands. In: NCDENR Stormwater BMP Manual. Raleigh (NC): North Carolina Department of Environmental Resources; 2009. [revised 2009 Jul 10; accessed 2023 Dec 22]. https://www.deq.nc.gov/water-quality/surface-water-protection/spu/spu-bmp-manual-documents/bmpman-ch09-swwetlands-20090710-dwq-spu/download.
- US EPA. Storm water technology fact sheet: bioretention. Washington (DC): United States Environmental Protection Agency; 1999 Sep [accessed 2023 Dec 22]. EPA 832-F-99-012. https://nepis.epa.gov/Exe/tiff2png.cgi/200044BE.PNG?-r+85+-g+15+D%3A%5CZYFILES%5CINDEX%20DATA%5C95THRU99%5CTIFF%5C00001323%5C200044BE.TIF.
- US EPA. Storm water technology fact sheet: storm water wetlands. Washington (DC): United States Environmental Protection Agency; 1999. [accessed 2023 Dec 22]. EPA 832-F-99-025. https://nepis.epa.gov/Exe/ZyNET.exe/200044BW.TXT?ZyActionD=ZyDocument&Client=EPA&Index=1995+Thru+1999&Docs=&Query=&Time=&EndTime=&SearchMethod=1&TocRestrict=n&Toc=&TocEntry=&QField=&QFieldYear=&QFieldMonth=&QFieldDay=&IntQFieldOp=0&ExtQFieldOp=0&XmlQuery=&File=D%3A%5Czyfiles%5CIndex%20Data%5C95thru99%5CTxt%5C00000015%5C200044BW.txt&User=ANONYMOUS&Password=anonymous&SortMethod=h%7C-&MaximumDocuments=1&FuzzyDegree=0&ImageQuality=r75g8/r75g8/x150y150g16/i425&Display=hpfr&DefSeekPage=x&SearchBack=ZyActionL&Back=ZyActionS&BackDesc=Results%20page&MaximumPages=1&ZyEntry=1&SeekPage=x&ZyPURL
- Ahammed F. A review of water-sensitive urban design technologies and practices for sustainable stormwater management. Sustainable Water Resources Management. 2017 Sep [accessed 2023 Dec 22];3:269–282. doi:10.1007/s40899-017-0093-8.
- Dutta A, Torres A, Vojinovic Z. Evaluation of pollutant removal efficiency by small-scale nature-based solutions focusing on bio-retention cells, vegetative swale and porous pavement. Water. 2021 Aug [accessed 2023 Dec 22];13(17):2361. doi:10.3390/w13172361.
Additional Resources
Davis P, Park D. Native Plant Selection for Out-of-Play Areas of South Carolina Golf Courses. Clemson (SC): Clemson Cooperative Extension, Land-Grant Press by Clemson Extension; 2022 Jun. LGP 1147. https://lgpress.clemson.edu/publication/native-plant-selection-for-out-of-play-areas-of-south-carolina-golf-courses/.